-
- News
- Books
Featured Books
- design007 Magazine
Latest Issues
Current IssueShowing Some Constraint
A strong design constraint strategy carefully balances a wide range of electrical and manufacturing trade-offs. This month, we explore the key requirements, common challenges, and best practices behind building an effective constraint strategy.
All About That Route
Most designers favor manual routing, but today's interactive autorouters may be changing designers' minds by allowing users more direct control. In this issue, our expert contributors discuss a variety of manual and autorouting strategies.
Creating the Ideal Data Package
Why is it so difficult to create the ideal data package? Many of these simple errors can be alleviated by paying attention to detail—and knowing what issues to look out for. So, this month, our experts weigh in on the best practices for creating the ideal design data package for your design.
- Articles
- Columns
- Links
- Media kit
||| MENU - design007 Magazine
Estimated reading time: 9 minutes
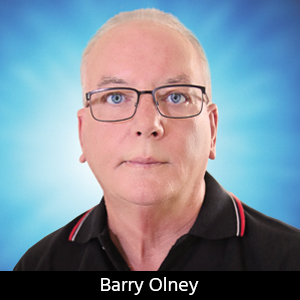
Beyond Design: The Coupling Coup
Coupling on a multilayer PCB may be a good or bad thing. On one hand, close coupling of signal traces to reference planes and differential pair signals is the best way to prevent common mode radiation and to mitigate electromagnetic (EM) emission. But on the other hand, close coupling of unrelated signal traces can bring us grief with unintentional crosstalk caused by overlapping EM fields. In this month’s column, I will look at where close coupling should be used and where it should be avoided.
There are five common situations where coupling can influence the signal and power integrity of a design:
- Signal to reference plane coupling.
- Return path loop area.
- Planar coupling.
- Differential pair signal coupling.
- Induced crosstalk.
1. Signal-to-reference plane coupling
The first rule of stackup design is that all signal layers should be adjacent to, and closely coupled to, an uninterrupted reference plane, which creates a clear return path. Closely coupling a signal trace to the reference plane minimizes the loop area and reduces inductance. Also, reducing the dielectric height will result in a large reduction in crosstalk without having a negative impact on available space on your board. However, one must be aware that reducing the dielectric height will require an increase in trace width to accommodate the desired impedance of the transmission line. This could become an issue for a densely routed design. So, typically a ~3 mil thick dielectric is selected for microstrip configurations. Figure 1 illustrates the variation of dielectric thickness and trace width. For stripline configurations, the dielectric thickness generally falls between 4–5 mils due to the proximity of the two planes.
2. Return path loop area
Return path discontinuities (RPDs) can create large loop areas that increase series inductance, degrade signal integrity, and increase crosstalk and electromagnetic radiation. Small discontinuities, such as vias and non-uniform return paths on a bus, are becoming an important factor for the signal integrity and timing of high-speed systems. RPDs produce impedance discontinuities due to the local return inductance and capacitive changes. Impedance discontinuities create reflected noise, contribute to differential channel to channel noise, and may promote mode conversion.
Common mode radiation is the result of parasitics in the circuit that emanate from the unwanted voltage drops in the conductors. As the signal follows the transmission line, capacitive coupling between the trace and plane conductors completes the loop and displacement current flows through the capacitance which returns to the source. The common mode current that flows through the ground impedance produces a voltage drop in the digital logic ground system and generates magnetic radiation.
To control common mode radiation, it is important to minimize the common mode ground voltage at the source. Also, good grounding minimizes noise sources by presenting common mode currents with a low impedance path to ground potential. Returning signal currents tend to stay near their signal conductors, falling off in intensity with the square of increasing distance. If the return path of a common mode current is far from the signal path, then the common mode current will radiate. However, if you engineer the return path to be near the source current, then the loop area will be small and therefore the common mode current will not radiate.
3. Planar coupling
With the continuous trend to smaller feature sizes and faster signal speeds, planar capacitor laminate or embedded capacitor materials (ECM) are becoming a cost-effective solution for improved power integrity. This technology provides an effective approach for decoupling high-performance ICs whilst also reducing electromagnetic interference. Coupling the planes very close together creates high capacitance.
Embedded capacitance technology allows for a very thin dielectric layer (0.24–2.0 mil) that provides distributive decoupling capacitance and takes the place of conventional discrete decoupling capacitors over 1 GHz. Unfortunately, standard decoupling capacitors have little effect over 1 GHz and the only way to reduce the AC impedance of the power distribution network above this frequency is to use ECM or, alternatively, die capacitance. These ultra-thin laminates replace the conventional power and ground planes and have excellent stability of dielectric constant and loss up to 15 GHz.
Planar capacitance requires a very thin dielectric with a high dielectric constant (Dk). This is contrary to the typical high-speed design that requires a low dielectric constant to improve dissipation losses. However, in this case, we need high capacitance, hence the high Dk. With 20 nF per square inch in capacitance density, 3M ECM is the highest capacitance density embedded-capacitance material on the market (Table 1).
4. Differential Pair Signal Coupling
Electromagnetic radiation from digital circuits can occur as either differential mode or common mode. Differential mode is typically equal and opposite, and therefore any radiating fields will cancel. Conversely, common mode radiation from two coupled conductors is identical. It does not cancel but rather reinforces. Unfortunately, differential mode propagation can be converted to common mode by parasitic capacitance, or any imbalance caused by signal skew, rise/fall time mismatch, or asymmetry in the channel. Also, return path discontinuities can create large common-mode loop areas that increase series inductance and electromagnetic radiation.
In the case of differential pairs, the transformation from differential mode to common mode typically takes place on bends and non-symmetrical routing, near via and pin obstructions, but can also be caused by small changes in impedance due to RPDs.
If a differential pair is well balanced, then tight coupling will achieve an effective degree of field cancellation. However, if they are not perfectly balanced (Figure 2), then the degree of cancellation is not determined by the spacing, but rather by the common-mode balance of the differential pair. Most digital drivers have poor common-mode balance and therefore differential pairs often radiate far more power in the common mode than in the differential mode. In such a case, one gains no radiation benefit from coupling the differential traces more closely together.
Differential signals that are closely coupled will operate mainly in the differential mode with some common-mode radiation from imbalances in the signals. If the two traces are separated enough to prevent coupling, then both act as single-ended signals. So, a 100-ohm differential pair becomes two individual 50 ohm single-ended signals. This is fine, providing the loop area is small and the impedance does not change along the length of the signals.
5. Induced Crosstalk
As signal traces come into proximity of an aggressor signal, part of that signal is unintentionally electromagnetically coupled into the victim trace as noise due to the overlapping of EM fields. Crosstalk is three-dimensional and is dependent on the signal trace separation, the trace to plane(s) separation, parallel segment length, the transmission line load, and the technology employed. But crosstalk also varies depending on the physical stackup configuration.
Crosstalk is caused by the coupling of electromagnetic fields. Electric fields cause signal voltages to capacitively couple into nearby traces. Capacitive coupling draws a surge of drive current which causes reflections on the transmission lines. Whereas magnetic fields cause signal currents to be induced into nearby traces, inductive coupling produces ground bounce and power supply noise. Crosstalk falls off rapidly with the square of the distance. The degree of impact is related to the aggressor signal voltage, available board real estate, and thus the proximity of signal traces.
The easiest way to reduce crosstalk from a nearby aggressor signal is, of course, to increase the spacing between the signals in question. Doubling the spacing cuts the crosstalk to roughly a quarter of its original level. However, crosstalk is determined by the ratio of the trace separation and the height of the trace above the plane. By varying the trace height, one can also control the coupling—hence crosstalk. If real estate is limited, then this may be a better solution rather than increasing routing density. A tight coupling (less height) results in less crosstalk.
There is a sweet spot where the total energy stored in the electromagnetic field surrounding the trace is optimized. Crosstalk between two or more conductors depends on their mutual inductance and mutual capacitance. The inductance plays a major role in this coupling. The signal return currents will generate EM fields. Those EM fields, in turn, induce voltages (crosstalk) into other signals.
It can be seen in Figure 3 that the differential impedance or the coupling of two parallel traces levels off at 100? above 12 mils trace clearance (blue curve). This is simulated quickly by multiple passes of the field solver. All other factors being equal, the differential impedance will always be 100? regardless of increased spacing. This also represents the point at which crosstalk (coupling) begins. This curve provides a clear map of the design space and efficiently defines the stackup configuration for single-ended and coupled pairs. In this case, once the separation is less than 12 mils, the two traces begin to couple and transfer electromagnetic energy.
Crosstalk is typically picked up on long parallel trace segments. These can be on the same layer but may also be broadside-coupled from the adjacent layer. It is for this reason that orthogonal routing is recommended on adjacent layers (between planes) to minimize the coupling area.
In conclusion, parasitic effects can be minimized by separating traces as much as possible, coupling signal traces close to the reference planes, reducing the loop area of return current, using good stackup design practices, and by lowing the AC impedance of the PDN by using distributed embedded capacitance.
Key Points:
- The first rule of stackup design is that all signal layers should be adjacent to, and closely coupled to, an uninterrupted reference plane, which creates a clear return path.
- Return path discontinuities (RPDs) can create large loop areas that increase series inductance, degrade signal integrity, and increase crosstalk and electromagnetic radiation.
- Common mode radiation is the result of parasitics in the circuit that emanate from the unwanted voltage drops in the conductors.
- If the return path of a common mode current is far from the signal path, then the common mode current will radiate.
- ECM technology provides an effective approach for decoupling high-performance ICs whilst also reducing electromagnetic interference. Coupling the planes very close together creates high capacitance.
- ECM provide distributive decoupling capacitance that take the place of conventional discrete decoupling capacitors over 1 GHz.
- Differential mode propagation can be converted to common mode by parasitic capacitance or any imbalance caused by signal skew, rise/fall time mismatch, or asymmetry in the channel.
- In the case of differential pairs, the transformation from differential mode to common mode typically takes place on bends and non-symmetrical routing, near via and pin obstructions, but can also be caused by small changes in impedance due to RPDs.
- Differential signals that are closely coupled will operate mainly in the differential mode with some common-mode radiation from imbalances in the signals.
- If the two traces of a differential pair are separated enough, to prevent coupling, then both act as single-ended signals.
- As signal traces come into proximity of an aggressor signal, part of that signal is unintentionally electromagnetically coupled into the victim trace as noise, due to the overlapping of EM fields.
- The easiest way to reduce crosstalk from a nearby aggressor signal is, of course, to increase the spacing between the signals in question.
- By varying the trace height, one can also control the coupling—hence crosstalk. If real estate is limited, then this may be a better solution.
- Crosstalk is typically picked up on long parallel trace segments. These can be on the same layer but may also be broadside-coupled from the adjacent layer.
Columns by Barry Olney:
- “Stackup Configurations to Mitigate Crosstalk”
- “The Dark Side—Return of the Signal”
- “Stackup Planning, Part 1”
- “The Curse of the Golden Board”
- “Material Selection for SERDES Design”
- “Common Symptoms of Common Mode Radiation”
This column originally appeared in the March 2022 issue of Design007 Magazine.
More Columns from Beyond Design
Beyond Design: Refining Design ConstraintsBeyond Design: The Metamorphosis of the PCB Router
Beyond Design: Radiation and Interference Coupling
Beyond Design: Key SI Considerations for High-speed PCB Design
Beyond Design: Electro-optical Circuit Boards
Beyond Design: AI-driven Inverse Stackup Optimization
Beyond Design: High-speed Rules of Thumb
Beyond Design: Integrated Circuit to PCB Integration